A molecular and cellular perspective on human brain evolution and tempo
The evolution of the modern human brain was accompanied by distinct molecular and cellular specializations, which underpin our diverse cognitive abilities but also increase our susceptibility to neurological diseases. These features, some specific to humans and others shared with related species, manifest during different stages of brain development. In this multi-stage process, neural stem cells proliferate to produce a large and diverse progenitor pool, giving rise to excitatory or inhibitory neurons that integrate into circuits during further maturation. This process unfolds over varying time scales across species and has progressively become slower in the human lineage, with differences in tempo correlating with differences in brain size, cell number and diversity, and connectivity. Here we introduce the terms ‘bradychrony’ and ‘tachycrony’ to describe slowed and accelerated developmental tempos, respectively. We review how recent technical advances across disciplines, including advanced engineering of in vitro models, functional comparative genetics and high-throughput single-cell profiling, are leading to a deeper understanding of how specializations of the human brain arise during bradychronic neurodevelopment. Emerging insights point to a central role for genetics, gene-regulatory networks, cellular innovations and developmental tempo, which together contribute to the establishment of human specializations during various stages of neurodevelopment and at different points in evolution.
This is a preview of subscription content, access via your institution
Access options
Access Nature and 54 other Nature Portfolio journals
Get Nature+, our best-value online-access subscription
cancel any time
Subscribe to this journal
Receive 51 print issues and online access
196,21 € per year
only 3,85 € per issue
Buy this article
- Purchase on SpringerLink
- Instant access to full article PDF
Prices may be subject to local taxes which are calculated during checkout
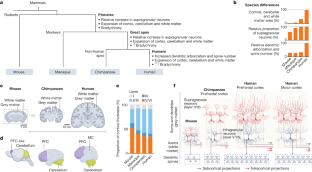

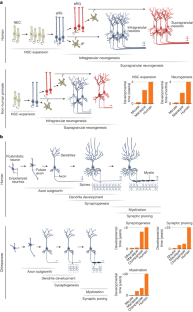
Similar content being viewed by others
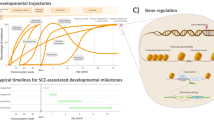
Cortical cellular diversity and development in schizophrenia
Article 13 May 2020
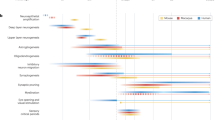
Human neuronal maturation comes of age: cellular mechanisms and species differences
Article 23 November 2023
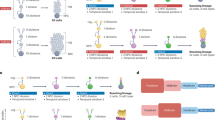
Indirect neurogenesis in space and time
Article 01 July 2024
References
- Lage, C. A., Wolmarans, D. W. & Mograbi, D. C. An evolutionary view of self-awareness. Behav. Process.194, 104543 (2022). ArticleGoogle Scholar
- Roth, G. & Dicke, U. Origin and evolution of human cognition. Prog. Brain Res.250, 285–316 (2019). ArticlePubMedGoogle Scholar
- Mouritsen, H., Heyers, D. & Güntürkün, O. The neural basis of long-distance navigation in birds. Annu. Rev. Physiol.78, 133–154 (2015). ArticlePubMedGoogle Scholar
- Jarvis, E. D. Evolution of vocal learning and spoken language. Science366, 50–54 (2019). ArticleADSCASPubMedGoogle Scholar
- Whiten, A., Hinde, R. A., Laland, K. N. & Stringer, C. B. Culture evolves. Philos. Trans. R. Soc. B366, 938–948 (2011). ArticleGoogle Scholar
- Varki, A. & Gagneux, P. in On Human Nature: Biology, Psychology, Ethics, Politics, and Religion (eds Tibayrenc, M. et al.) 151–160 (Academic, 2017).
- Pattabiraman, K., Muchnik, S. K. & Sestan, N. The evolution of the human brain and disease susceptibility. Curr. Opin. Genet. Dev.65, 91–97 (2020). ArticleCASPubMedGoogle Scholar
- Martinez, P. & Sprecher, S. G. Of circuits and brains: the origin and diversification of neural architectures. Front. Ecol. Evol.8, 82 (2020). ArticleGoogle Scholar
- Martín-Durán, J. M. & Hejnol, A. A developmental perspective on the evolution of the nervous system. Dev. Biol.475, 181–192 (2019). ArticlePubMedGoogle Scholar
- Vallender, E. J. Genetics of human brain evolution. Prog. Brain Res.250, 3–39 (2019). ArticlePubMedGoogle Scholar
- Uzquiano, A. & Arlotta, P. Brain organoids: the quest to decipher human-specific features of brain development. Curr. Opin. Genet. Dev.75, 101955 (2022). ArticleCASPubMedGoogle Scholar
- Vinsland, E. & Linnarsson, S. Single-cell RNA-sequencing of mammalian brain development: insights and future directions. Development149, dev200180 (2022). ArticleCASPubMedGoogle Scholar
- Kelley, K. W. & Pașca, S. P. Human brain organogenesis: toward a cellular understanding of development and disease. Cell185, 42–61 (2022). ArticleCASPubMedGoogle Scholar
- Perkel, J. M. Single-cell analysis enters the multiomics age. Nature595, 614–616 (2021). ArticleADSCASGoogle Scholar
- Chiaradia, I. & Lancaster, M. A. Brain organoids for the study of human neurobiology at the interface of in vitro and in vivo. Nat. Neurosci.23, 1496–1508 (2020). ArticleCASPubMedGoogle Scholar
- Benito-Kwiecinski, S. & Lancaster, M. A. Brain organoids: human neurodevelopment in a dish. Cold Spring Harb. Perspect. Biol.12, a035709 (2019). ArticleGoogle Scholar
- Pollen, A. A. et al. Establishing cerebral organoids as models of human-specific brain evolution. Cell176, 743–756.e17 (2019). ArticleCASPubMedPubMed CentralGoogle Scholar
- Jerison, H. J. The theory of encephalization. Ann. NY Acad. Sci.299, 146–160 (1977). ArticleADSCASPubMedGoogle Scholar
- Beaudet, A., Du, A. & Wood, B. Evolution of the modern human brain. Prog. Brain Res.250, 219–250 (2019). ArticlePubMedGoogle Scholar
- Du, A. et al. Pattern and process in hominin brain size evolution are scale-dependent. Proc. R. Soc. B285, 20172738 (2018). ArticlePubMedPubMed CentralGoogle Scholar
- Tilot, A. K. et al. The evolutionary history of common genetic variants influencing human cortical surface area. Cereb. Cortex31, 1873–1887 (2020). ArticlePubMed CentralGoogle Scholar
- Finlay, B. L. & Darlington, R. B. Linked regularities in the development and evolution of mammalian brains. Science268, 1578–1584 (1995). ArticleADSCASPubMedGoogle Scholar
- Finlay, B. L., Darlington, R. B. & Nicastro, N. Developmental structure in brain evolution. Behav. Brain Sci.24, 263–278 (2001). ArticleCASPubMedGoogle Scholar
- Montgomery, S. H., Mundy, N. I. & Barton, R. A. Brain evolution and development: adaptation, allometry and constraint. Proc. R. Soc. B283, 20160433 (2016). ArticlePubMedPubMed CentralGoogle Scholar
- Dunbar, R. I. M. Neocortex size as a constraint on group size in primates. J. Hum. Evol.22, 469–493 (1992). ArticleGoogle Scholar
- Dicke, U. & Roth, G. Neuronal factors determining high intelligence. Philos. Trans. R. Soc B371, 20150180 (2016). ArticleGoogle Scholar
- Herculano-Houzel, S. The human brain in numbers: a linearly scaled-up primate brain. Front. Hum. Neurosci.3, 31 (2009). ArticlePubMedPubMed CentralGoogle Scholar
- Azevedo, F. A. C. et al. Equal numbers of neuronal and nonneuronal cells make the human brain an isometrically scaled‐up primate brain. J. Comp. Neurol.513, 532–541 (2009). ArticlePubMedGoogle Scholar
- Gómez-Robles, A., Hopkins, W. D., Schapiro, S. J. & Sherwood, C. C. Relaxed genetic control of cortical organization in human brains compared with chimpanzees. Proc. Natl Acad. Sci. USA112, 14799–14804 (2015). ArticleADSPubMedPubMed CentralGoogle Scholar
- Hill, J. et al. Similar patterns of cortical expansion during human development and evolution. Proc. Natl Acad. Sci. USA107, 13135–13140 (2010). ArticleADSCASPubMedPubMed CentralGoogle Scholar
- Arnsten, A. F. T. Stress signalling pathways that impair prefrontal cortex structure and function. Nat. Rev. Neurosci.10, 410–422 (2009). ArticleCASPubMedPubMed CentralGoogle Scholar
- Holmes, A. J. et al. Prefrontal functioning during context processing in schizophrenia and major depression: An event-related fMRI study. Schizophr. Res.76, 199–206 (2005). ArticlePubMedGoogle Scholar
- Rougier, N. P., Noelle, D. C., Braver, T. S., Cohen, J. D. & O’Reilly, R. C. Prefrontal cortex and flexible cognitive control: rules without symbols. Proc. Natl Acad. Sci. USA102, 7338–7343 (2005). ArticleADSCASPubMedPubMed CentralGoogle Scholar
- León, M. S. Pde et al. The primitive brain of early Homo. Science372, 165–171 (2021). ArticleADSGoogle Scholar
- Sereno, M. I. et al. The human cerebellum has almost 80% of the surface area of the neocortex. Proc. Natl Acad. Sci. USA117, 19538–19543 (2020). ArticleADSCASPubMedPubMed CentralGoogle Scholar
- Buckner, R. L., Krienen, F. M., Castellanos, A., Diaz, J. C. & Yeo, B. T. T. The organization of the human cerebellum estimated by intrinsic functional connectivity. J. Neurophysiol.106, 2322–2345 (2011). ArticlePubMedPubMed CentralGoogle Scholar
- Balsters, J. H. et al. Evolution of the cerebellar cortex: the selective expansion of prefrontal-projecting cerebellar lobules. Neuroimage49, 2045–2052 (2010). ArticleCASPubMedGoogle Scholar
- Sultan, F. & Braitenberg, V. Shapes and sizes of different mammalian cerebella. A study in quantitative comparative neuroanatomy. J. Hirnforsch.34, 79–92 (1993). CASPubMedGoogle Scholar
- Zhang, K. & Sejnowski, T. J. A universal scaling law between gray matter and white matter of cerebral cortex. Proc. Natl Acad. Sci. USA97, 5621–5626 (2000). ArticleADSCASPubMedPubMed CentralGoogle Scholar
- Schoenemann, P. T., Sheehan, M. J. & Glotzer, L. D. Prefrontal white matter volume is disproportionately larger in humans than in other primates. Nat. Neurosci.8, 242–252 (2005). A cross-species analysis of 11 primate species, including human and other great apes, shows a profound disproportionate increase of relative prefrontal white matter volume in humans. ArticleCASPubMedGoogle Scholar
- Donahue, C. J., Glasser, M. F., Preuss, T. M., Rilling, J. K. & Essen, D. C. V. Quantitative assessment of prefrontal cortex in humans relative to nonhuman primates. Proc. Natl Acad. Sci. USA115, E5183–E5192 (2018). ArticleADSCASPubMedPubMed CentralGoogle Scholar
- von Economo, C. Cellular Structure of the Human Cerebral Cortex (Karger, 2009).
- Preuss, T. M. & Wise, S. P. Evolution of prefrontal cortex. Neuropsychopharmacology47, 3–19 (2022). ArticlePubMedGoogle Scholar
- Hutsler, J. J., Lee, D.-G. & Porter, K. K. Comparative analysis of cortical layering and supragranular layer enlargement in rodent carnivore and primate species. Brain Res.1052, 71–81 (2005). ArticleCASPubMedGoogle Scholar
- Balaram, P. & Kaas, J. H. Towards a unified scheme of cortical lamination for primary visual cortex across primates: insights from NeuN and vGLUT2 immunoreactivity. Front. Neuroanat.8, 81 (2014). ArticlePubMedPubMed CentralGoogle Scholar
- Sousa, A. Ade. et al. Comparative cytoarchitectural analyses of striate and extrastriate areas in hominoids. Cereb. Cortex20, 966–981 (2010). Refs. 44 and 46 show that a relative increase of supragranular layers is observed in great apes compared with other primates, but is not further increased in humans compared with other apes. ArticlePubMedGoogle Scholar
- Berg, J. et al. Human neocortical expansion involves glutamatergic neuron diversification. Nature598, 151–158 (2021). ArticleADSCASPubMedPubMed CentralGoogle Scholar
- Loomba, S. et al. Connectomic comparison of mouse and human cortex. Science377, eabo0924 (2022). ArticleCASPubMedGoogle Scholar
- Bakken, T. E. et al. Comparative cellular analysis of motor cortex in human, marmoset and mouse. Nature598, 111–119 (2021). ArticleADSCASPubMedPubMed CentralGoogle Scholar
- Jorstad, N. L. et al. Comparative transcriptomics reveals human-specific cortical features. Science382, eade9516 (2023). ArticleCASPubMedPubMed CentralGoogle Scholar
- Krienen, F. M. et al. Innovations present in the primate interneuron repertoire. Nature586, 262–269 (2020). This study shows that the ivy cell, an interneuron subtype, is present in the cortex and hippocampus in primates, but localizes to the hippocampus only in mice and ferrets. ArticleADSCASPubMedPubMed CentralGoogle Scholar
- Cerminara, N. L., Lang, E. J., Sillitoe, R. V. & Apps, R. Redefining the cerebellar cortex as an assembly of non-uniform Purkinje cell microcircuits. Nat. Rev. Neurosci.16, 79–93 (2015). ArticleCASPubMedPubMed CentralGoogle Scholar
- Kozareva, V. et al. A transcriptomic atlas of mouse cerebellar cortex comprehensively defines cell types. Nature598, 214–219 (2021). ArticleADSCASPubMedPubMed CentralGoogle Scholar
- Haldipur, P. et al. Spatiotemporal expansion of primary progenitor zones in the developing human cerebellum. Science366, 454–460 (2019). ArticleADSCASPubMedPubMed CentralGoogle Scholar
- Falcone, C. et al. Redefining varicose projection astrocytes in primates. Glia70, 145–154 (2022). ArticlePubMedGoogle Scholar
- Geirsdottir, L. et al. Cross-species single-cell analysis reveals divergence of the primate microglia program. Cell179, 1609–1622.e16 (2019). ArticleCASPubMedGoogle Scholar
- Masuda, T. et al. Spatial and temporal heterogeneity of mouse and human microglia at single-cell resolution. Nature566, 388–392 (2019). ArticleADSCASPubMedGoogle Scholar
- Berto, S. et al. Accelerated evolution of oligodendrocytes in the human brain. Proc. Natl Acad. Sci. USA116, 24334–24342 (2019). ArticleADSCASPubMedPubMed CentralGoogle Scholar
- Oberheim, N. A. et al. Uniquely hominid features of adult human astrocytes. J. Neurosci.29, 3276–3287 (2009). ArticleCASPubMedPubMed CentralGoogle Scholar
- Caglayan, E. et al. Molecular features driving cellular complexity of human brain evolution. Nature620, 145–153 (2023). ArticleADSCASPubMedPubMed CentralGoogle Scholar
- Fang, R. et al. Conservation and divergence of cortical cell organization in human and mouse revealed by MERFISH. Science377, 56–62 (2022). ArticleADSCASPubMedPubMed CentralGoogle Scholar
- Hodge, R. D. et al. Conserved cell types with divergent features in human versus mouse cortex. Nature573, 61–68 (2019). ArticleADSCASPubMedPubMed CentralGoogle Scholar
- Sousa, A. M. M. et al. Molecular and cellular reorganization of neural circuits in the human lineage. Science358, 1027–1032 (2017). ArticleADSCASPubMedPubMed CentralGoogle Scholar
- Schmitz, M. T. et al. The development and evolution of inhibitory neurons in primate cerebrum. Nature603, 871–877 (2022). ArticleADSCASPubMedPubMed CentralGoogle Scholar
- Ardesch, D. J. et al. Evolutionary expansion of connectivity between multimodal association areas in the human brain compared with chimpanzees. Proc. Natl Acad. Sci. USA116, 201818512 (2019). ArticleGoogle Scholar
- Bianchi, S. et al. Dendritic morphology of pyramidal neurons in the chimpanzee neocortex: regional specializations and comparison to humans. Cereb. Cortex23, 2429–2436 (2013). This study demonstrates that pyramidal neurons in different neocortical structures exhibit increased dendritic arborization and synapse number per neuron in humans compared with chimpanzees. ArticlePubMedGoogle Scholar
- Mohan, H. et al. Dendritic and axonal architecture of individual pyramidal neurons across layers of adult human neocortex. Cereb. Cortex25, 4839–4853 (2015). ArticlePubMedPubMed CentralGoogle Scholar
- Elston, G. N. et al. Specializations of the granular prefrontal cortex of primates: implications for cognitive processing. Anat. Rec. A288A, 26–35 (2006). ArticleGoogle Scholar
- Elston, G. N., Benavides-Piccione, R. & DeFelipe, J. A study of pyramidal cell structure in the cingulate cortex of the macaque monkey with comparative notes on inferotemporal and primary visual cortex. Cereb. Cortex15, 64–73 (2005). ArticlePubMedGoogle Scholar
- Elston, G. N., Benavides-Piccione, R. & DeFelipe, J. The pyramidal cell in cognition: a comparative study in human and monkey. J. Neurosci.21, RC163 (2001). ArticleCASPubMedPubMed CentralGoogle Scholar
- Jacobs, B. et al. Regional dendritic and spine variation in human cerebral cortex: a quantitative Golgi study. Cereb. Cortex11, 558–571 (2001). ArticleCASPubMedGoogle Scholar
- Koopmans, F. et al. Comparative hippocampal synaptic proteomes of rodents and primates: differences in neuroplasticity-related proteins. Front. Mol. Neurosci.11, 364 (2018). ArticleCASPubMedPubMed CentralGoogle Scholar
- Beaulieu-Laroche, L. et al. Allometric rules for mammalian cortical layer 5 neuron biophysics. Nature600, 274–278 (2021). ArticleADSCASPubMedGoogle Scholar
- Gidon, A. et al. Dendritic action potentials and computation in human layer 2/3 cortical neurons. Science367, 83–87 (2020). ArticleADSCASPubMedGoogle Scholar
- Beaulieu-Laroche, L. et al. Enhanced dendritic compartmentalization in human cortical neurons. Cell175, 643–651.e14 (2018). ArticleCASPubMedPubMed CentralGoogle Scholar
- Eyal, G. et al. Unique membrane properties and enhanced signal processing in human neocortical neurons. eLife5, e16553 (2016). ArticlePubMedPubMed CentralGoogle Scholar
- Lee, B. R. et al. Signature morphoelectric properties of diverse GABAergic interneurons in the human neocortex. Science382, eadf6484 (2023). ArticleCASPubMedGoogle Scholar
- Chartrand, T. et al. Morphoelectric and transcriptomic divergence of the layer 1 interneuron repertoire in human versus mouse neocortex. Science382, eadf0805 (2023). ArticleCASPubMedGoogle Scholar
- Molnár, G. et al. Human pyramidal to interneuron synapses are mediated by multi-vesicular release and multiple docked vesicles. eLife5, e18167 (2016). ArticlePubMedPubMed CentralGoogle Scholar
- Libé-Philippot, B. et al. LRRC37B is a species-specific regulator of voltage-gated channels and excitability in human cortical neurons. Cell186, 5766–5783.e25 (2023). ArticlePubMedPubMed CentralGoogle Scholar
- Masoli, S. et al. Human outperform mouse Purkinje cells in dendritic complexity and computational capacity. Commun. Biol.7, 5 (2024). This comparative study shows that Purkinje cells exhibit increased dendritic arborization and spine numbers in humans compared with rodents. ArticleCASPubMedPubMed CentralGoogle Scholar
- DeSilva, J. & Lesnik, J. Chimpanzee neonatal brain size: Implications for brain growth in Homo erectus. J. Hum. Evol.51, 207–212 (2006). ArticlePubMedGoogle Scholar
- DeSilva, J. M. & Lesnik, J. J. Brain size at birth throughout human evolution: a new method for estimating neonatal brain size in hominins. J. Hum. Evol.55, 1064–1074 (2008). ArticlePubMedGoogle Scholar
- Sakai, T. et al. Differential prefrontal white matter development in chimpanzees and humans. Curr. Biol.22, 171 (2012). ArticleCASGoogle Scholar
- Kollmann, J. Das Ueberwintern von europäischen Frosch- und Tritonlarven und die Umwandlung des mexikanischen Axolotl. Verh. Naturforsch. Ges. Basel7, 387–398 (1885). Google Scholar
- Berry, R. J. & Bronson, F. H. Life history and bioeconomy of the house mouse. Biol. Rev.67, 519–550 (1992). ArticleCASPubMedGoogle Scholar
- Guyer, A. E., Pérez‐Edgar, K. & Crone, E. A. Opportunities for neurodevelopmental plasticity from infancy through early adulthood. Child Dev.89, 687–697 (2018). ArticlePubMedPubMed CentralGoogle Scholar
- Libé-Philippot, B. & Vanderhaeghen, P. Cellular and molecular mechanisms linking human cortical development and evolution. Annu Rev Genet55, 555–581 (2021). ArticlePubMedGoogle Scholar
- Villalba, A., Götz, M. & Borrell, V. The regulation of cortical neurogenesis. Curr. Top. Dev. Biol.142, 1–66 (2020). CASPubMedGoogle Scholar
- Miller, D. J., Bhaduri, A., Sestan, N. & Kriegstein, A. Shared and derived features of cellular diversity in the human cerebral cortex. Curr. Opin. Neurobiol.56, 117–124 (2019). ArticleCASPubMedPubMed CentralGoogle Scholar
- Stepien, B. K., Vaid, S. & Huttner, W. B. Length of the neurogenic period—a key determinant for the generation of upper-layer neurons during neocortex development and evolution. Front. Cell Dev. Biol.9, 676911 (2021). ArticlePubMedPubMed CentralGoogle Scholar
- Silbereis, J. C., Pochareddy, S., Zhu, Y., Li, M. & Sestan, N. The cellular and molecular landscapes of the developing human central nervous system. Neuron89, 248–268 (2016). ArticleCASPubMedPubMed CentralGoogle Scholar
- Haubensak, W., Attardo, A., Denk, W. & Huttner, W. B. Neurons arise in the basal neuroepithelium of the early mammalian telencephalon: a major site of neurogenesis. Proc. Natl Acad. Sci. USA101, 3196–3201 (2004). ArticleADSCASPubMedPubMed CentralGoogle Scholar
- Sakai, T. et al. Fetal brain development in chimpanzees versus humans. Curr. Biol.22, R791–R792 (2012). ArticleCASPubMedGoogle Scholar
- Otani, T., Marchetto, M. C., Gage, F. H., Simons, B. D. & Livesey, F. J. 2D and 3D stem cell models of primate cortical development identify species-specific differences in progenitor behavior contributing to brain size. Cell Stem Cell18, 467–480 (2016). ArticleCASPubMedPubMed CentralGoogle Scholar
- Wang, X., Tsai, J.-W., LaMonica, B. & Kriegstein, A. R. A new subtype of progenitor cell in the mouse embryonic neocortex. Nat. Neurosci.14, 555–561 (2011). ArticleCASPubMedPubMed CentralGoogle Scholar
- Hansen, D. V., Lui, J. H., Parker, P. R. L. & Kriegstein, A. R. Neurogenic radial glia in the outer subventricular zone of human neocortex. Nature464, 554–561 (2010). ArticleADSCASPubMedGoogle Scholar
- Kanton, S. et al. Organoid single-cell genomic atlas uncovers human-specific features of brain development. Nature574, 418–422 (2019). ArticleADSCASPubMedGoogle Scholar
- Mora-Bermúdez, F. et al. Differences and similarities between human and chimpanzee neural progenitors during cerebral cortex development. eLife5, e18683 (2016). ArticlePubMedPubMed CentralGoogle Scholar
- Benito-Kwiecinski, S. et al. An early cell shape transition drives evolutionary expansion of the human forebrain. Cell184, 2084–2102.e19 (2021). ArticleCASPubMedPubMed CentralGoogle Scholar
- Taverna, E., Götz, M. & Huttner, W. B. The cell biology of neurogenesis: toward an understanding of the development and evolution of the neocortex. Annu. Rev. Cell Dev. Biol.30, 465–502 (2014). ArticleCASPubMedGoogle Scholar
- O’Rahilly, R. & Müller, F. Developmental stages in human embryos: revised and new measurements. Cells Tissues Organs192, 73–84 (2010). ArticlePubMedGoogle Scholar
- Rakic, P. Specification of cerebral cortical areas. Science241, 170–176 (1988). ArticleADSCASPubMedGoogle Scholar
- Davignon, R. W., Parker, R. M. & Hendrickx, A. G. Staging of the early embryonic brain in the baboon (Papio cynocephalus) and rhesus monkey (Macaca mulatta). Anat. Embryol.159, 317–334 (1980). ArticleCASGoogle Scholar
- Pryor, S. E., Massa, V., Savery, D., Greene, N. D. E. & Copp, A. J. Convergent extension analysis in mouse whole embryo culture. Methods Mol. Biol.839, 133–146 (2012). ArticleCASPubMedPubMed CentralGoogle Scholar
- Ziffra, R. S. et al. Single-cell epigenomics reveals mechanisms of human cortical development. Nature598, 205–213 (2021). ArticleADSCASPubMedPubMed CentralGoogle Scholar
- Collins, C. E., Airey, D. C., Young, N. A., Leitch, D. B. & Kaas, J. H. Neuron densities vary across and within cortical areas in primates. Proc. Natl Acad. Sci. USA107, 15927–15932 (2010). ArticleADSCASPubMedPubMed CentralGoogle Scholar
- Smart, I. H. M., Dehay, C., Giroud, P., Berland, M. & Kennedy, H. Unique morphological features of the proliferative zones and postmitotic compartments of the neural epithelium giving rise to striate and extrastriate cortex in the monkey. Cereb. Cortex12, 37–53 (2002). ArticlePubMedGoogle Scholar
- Krienen, F. M. et al. A marmoset brain cell census reveals regional specialization of cellular identities. Sci. Adv.9, eadk3986 (2023). ArticleCASPubMedPubMed CentralGoogle Scholar
- Ma, T. et al. Subcortical origins of human and monkey neocortical interneurons. Nat. Neurosci.16, 1588–1597 (2013). ArticleCASPubMedGoogle Scholar
- Jakovcevski, I., Mayer, N. & Zecevic, N. Multiple origins of human neocortical interneurons are supported by distinct expression of transcription factors. Cereb. Cortex21, 1771–1782 (2011). ArticlePubMedGoogle Scholar
- Yu, X. & Zecevic, N. Dorsal radial glial cells have the potential to generate cortical interneurons in human but not in mouse brain. J. Neurosci.31, 2413–2420 (2011). ArticleCASPubMedPubMed CentralGoogle Scholar
- Petanjek, Z., Berger, B. & Esclapez, M. Origins of cortical GABAergic neurons in the cynomolgus monkey. Cereb. Cortex19, 249–262 (2008). ArticlePubMedPubMed CentralGoogle Scholar
- Letinic, K., Zoncu, R. & Rakic, P. Origin of GABAergic neurons in the human neocortex. Nature417, 645–649 (2002). ArticleADSCASPubMedGoogle Scholar
- Delgado, R. N. et al. Individual human cortical progenitors can produce excitatory and inhibitory neurons. Nature601, 397–403 (2022). ArticleADSCASPubMedGoogle Scholar
- Hansen, D. V. et al. Non-epithelial stem cells and cortical interneuron production in the human ganglionic eminences. Nat. Neurosci.16, 1576–1587 (2013). ArticleCASPubMedPubMed CentralGoogle Scholar
- Bandler, R. C. et al. Single-cell delineation of lineage and genetic identity in the mouse brain. Nature601, 404–409 (2022). ArticleADSCASPubMedGoogle Scholar
- Chung, C. et al. Cell-type-resolved mosaicism reveals clonal dynamics of the human forebrain. Nature629, 384–392 (2024). ArticleCASPubMedGoogle Scholar
- Bakken, T. E. et al. Single-cell and single-nucleus RNA-seq uncovers shared and distinct axes of variation in dorsal LGN neurons in mice, non-human primates, and humans. eLife10, e64875 (2021). ArticleCASPubMedPubMed CentralGoogle Scholar
- Arcelli, P., Frassoni, C., Regondi, M. C., Biasi, S. D. & Spreafico, R. GABAergic neurons in mammalian thalamus: a marker of thalamic complexity? Brain Res. Bull.42, 27–37 (1997). ArticleCASPubMedGoogle Scholar
- Jager, P. et al. Dual midbrain and forebrain origins of thalamic inhibitory interneurons. eLife10, e59272 (2021). ArticleCASPubMedPubMed CentralGoogle Scholar
- Jager, P. et al. Tectal-derived interneurons contribute to phasic and tonic inhibition in the visual thalamus. Nat. Commun.7, 13579 (2016). ArticleADSCASPubMedPubMed CentralGoogle Scholar
- Letinic, K. & Rakic, P. Telencephalic origin of human thalamic GABAergic neurons. Nat. Neurosci.4, 931–936 (2001). ArticleCASPubMedGoogle Scholar
- Rakić, P. & Sidman, R. L. Telencephalic origin of pulvinar neurons in the fetal human brain. Z. Anat. Entwickl. Gesch.129, 53–82 (1969). ArticleGoogle Scholar
- Wallace, J. L. & Pollen, A. A. Human neuronal maturation comes of age: cellular mechanisms and species differences. Nat. Rev. Neurosci.25, 7–29 (2024). ArticleCASPubMedGoogle Scholar
- Vanderhaeghen, P. & Polleux, F. Developmental mechanisms underlying the evolution of human cortical circuits. Nat. Rev. Neurosci.24, 213–232 (2023). ArticleCASPubMedPubMed CentralGoogle Scholar
- Revah, O. et al. Maturation and circuit integration of transplanted human cortical organoids. Nature610, 319–326 (2022). ArticleADSCASPubMedPubMed CentralGoogle Scholar
- Giandomenico, S. L. et al. Cerebral organoids at the air-liquid interface generate diverse nerve tracts with functional output. Nat. Neurosci.22, 669–679 (2019). ArticleCASPubMedPubMed CentralGoogle Scholar
- Linaro, D. et al. Xenotransplanted human cortical neurons reveal species-specific development and functional integration into mouse visual circuits. Neuron104, 972–986.e6 (2019). ArticleCASPubMedPubMed CentralGoogle Scholar
- Zhu, Y. et al. Spatiotemporal transcriptomic divergence across human and macaque brain development. Science362, eaat8077 (2018). ArticleADSCASPubMedPubMed CentralGoogle Scholar
- Wang, L. et al. A cross-species proteomic map reveals neoteny of human synapse development. Nature622, 112–119 (2023). ArticleADSCASPubMedPubMed CentralGoogle Scholar
- Wildenberg, G., Li, H. & Kasthuri, N. The development of synapses in mouse and macaque primary sensory cortices. Preprint at bioRxivhttps://doi.org/10.1101/2023.02.15.528564 (2023).
- Huttenlocher, P. R. & Dabholkar, A. S. Regional differences in synaptogenesis in human cerebral cortex. J. Comp. Neurol.387, 167–178 (1997). ArticleCASPubMedGoogle Scholar
- Rakic, P., Bourgeois, J.-P., Eckenhoff, M. F., Zecevic, N. & Goldman-Rakic, P. S. Concurrent overproduction of synapses in diverse regions of the primate cerebral cortex. Science232, 232–235 (1986). ArticleADSCASPubMedGoogle Scholar
- Zeiss, C. J. Comparative milestones in rodent and human postnatal central nervous system development. Toxicol. Pathol.49, 1368–1373 (2021). ArticlePubMedGoogle Scholar
- Meng, Y., Jiang, J., Bachevalier, J., Zhang, X. & Chan, A. W. S. Developmental whole brain white matter alterations in transgenic Huntington’s disease monkey. Sci. Rep.7, 379 (2017). ArticleADSPubMedPubMed CentralGoogle Scholar
- Sakai, T. et al. Developmental trajectory of the corpus callosum from infancy to the juvenile stage: comparative MRI between chimpanzees and humans. PLoS ONE12, e0179624 (2017). ArticlePubMedPubMed CentralGoogle Scholar
- Semple, B. D., Blomgren, K., Gimlin, K., Ferriero, D. M. & Noble-Haeusslein, L. J. Brain development in rodents and humans: identifying benchmarks of maturation and vulnerability to injury across species. Prog. Neurobiol.106, 1–16 (2013). ArticlePubMedGoogle Scholar
- Bourgeois, J. & Rakic, P. Changes of synaptic density in the primary visual cortex of the macaque monkey from fetal to adult stage. J. Neurosci.13, 2801–2820 (1993). ArticleCASPubMedPubMed CentralGoogle Scholar
- De Felipe, J., Marco, P., Fairén, A. & Jones, E. G. Inhibitory synaptogenesis in mouse somatosensory cortex. Cereb. Cortex7, 619–634 (1997). ArticleCASPubMedGoogle Scholar
- Schörnig, M. et al. Comparison of induced neurons reveals slower structural and functional maturation in humans than in apes. eLife10, e59323 (2021). ArticlePubMedPubMed CentralGoogle Scholar
- Lindhout, F. W. et al. Quantitative mapping of transcriptome and proteome dynamics during polarization of human iPSC-derived neurons. eLife9, e58124 (2020). This study reports the identification of an intermediate axon developmental stage in human neurons. ArticleCASPubMedPubMed CentralGoogle Scholar
- Dotti, C., Sullivan, C. & Banker, G. The establishment of polarity by hippocampal neurons in culture. J. Neurosci.8, 1454–1468 (1988). ArticleCASPubMedPubMed CentralGoogle Scholar
- Lindhout, F. W. et al. Centrosome‐mediated microtubule remodeling during axon formation in human iPSC‐derived neurons. EMBO J.40, e106798 (2021). This study shows that centrosomes modulate axon development in human neurons, with a human-specific localization of axon initial segment protein TRIM46 to centrosomes prior to axon formation. ArticleCASPubMedPubMed CentralGoogle Scholar
- Bianchi, S. et al. Synaptogenesis and development of pyramidal neuron dendritic morphology in the chimpanzee neocortex resembles humans. Proc. Natl Acad. Sci. USA110, 10395–10401 (2013). ArticleADSCASPubMedPubMed CentralGoogle Scholar
- Rayon, T. et al. Species-specific pace of development is associated with differences in protein stability. Science369, eaba7667 (2020). ArticleCASPubMedPubMed CentralGoogle Scholar
- Lázaro, J. et al. A stem cell zoo uncovers intracellular scaling of developmental tempo across mammals. Cell Stem Cell30, 938–949.e7 (2023). ArticlePubMedPubMed CentralGoogle Scholar
- Matsuda, M. et al. Species-specific segmentation clock periods are due to differential biochemical reaction speeds. Science369, 1450–1455 (2020). ArticleADSCASPubMedGoogle Scholar
- Iwata, R. et al. Mitochondria metabolism sets the species-specific tempo of neuronal development. Science379, eabn4705 (2023). In vitro experiments show that mitochondrial metabolism regulates species-specific neurodevelopmental tempo. ArticleCASPubMedGoogle Scholar
- Ciceri, G. et al. An epigenetic barrier sets the timing of human neuronal maturation. Nature 626626, 881–890 (2024). This study presents dentification of a set of chromatin modifiers orchestrating neuronal maturation tempo.ArticleCASGoogle Scholar
- Wang, J., Weatheritt, R. & Voineagu, I. Alu-minating the mechanisms underlying primate cortex evolution. Biol. Psychiatry92, 760–771 (2022). ArticlePubMedGoogle Scholar
- Nesta, A. V., Tafur, D. & Beck, C. R. Hotspots of human mutation. Trends Genet.37, 717–729 (2021). ArticleCASPubMedGoogle Scholar
- Suntsova, M. V. & Buzdin, A. A. Differences between human and chimpanzee genomes and their implications in gene expression, protein functions and biochemical properties of the two species. BMC Genomics21, 535 (2020). ArticleCASPubMedPubMed CentralGoogle Scholar
- Saitou, M. & Gokcumen, O. An evolutionary perspective on the impact of genomic copy number variation on human health. J. Mol. Evol.88, 104–119 (2020). ArticleADSCASPubMedGoogle Scholar
- Dennis, M. Y. & Eichler, E. E. Human adaptation and evolution by segmental duplication. Curr. Opin. Genet. Dev.41, 44–52 (2016). ArticleCASPubMedPubMed CentralGoogle Scholar
- Waterson, R. H., Lander, E. S. & Wilson, R. K. Initial sequence of the chimpanzee genome and comparison with the human genome. Nature437, 69–87 (2005). ArticleGoogle Scholar
- Nurk, S. et al. The complete sequence of a human genome. Science376, 44–53 (2022). ArticleADSCASPubMedPubMed CentralGoogle Scholar
- An, N. A. et al. De novo genes with an lncRNA origin encode unique human brain developmental functionality. Nat. Ecol. Evol.7, 264–278 (2023). PubMedPubMed CentralGoogle Scholar
- Vakirlis, N., Vance, Z., Duggan, K. M. & McLysaght, A. De novo birth of functional microproteins in the human lineage. Cell Rep.41, 111808 (2022). ArticleCASPubMedPubMed CentralGoogle Scholar
- Levchenko, A., Kanapin, A., Samsonova, A. & Gainetdinov, R. R. Human accelerated regions and other human-specific sequence variations in the context of evolution and their relevance for brain development. Genome Biol. Evol.10, 166–188 (2017). ArticlePubMed CentralGoogle Scholar
- Franchini, L. F. & Pollard, K. S. Genomic approaches to studying human-specific developmental traits. Development142, 3100–3112 (2015). ArticleCASPubMedGoogle Scholar
- Pinson, A. et al. Human TKTL1 implies greater neurogenesis in frontal neocortex of modern humans than Neanderthals. Science377, eabl6422 (2022). ArticleCASPubMedGoogle Scholar
- Suzuki, I. K. et al. Human-specific NOTCH2NL genes expand cortical neurogenesis through Delta/Notch regulation. Cell173, 1370–1384.e16 (2018). ArticleCASPubMedPubMed CentralGoogle Scholar
- Fiddes, I. T. et al. Human-specific NOTCH2NL genes affect Notch signaling and cortical neurogenesis. Cell173, 1356–1369.e22 (2018). ArticleCASPubMedPubMed CentralGoogle Scholar
- Schmidt, E. R. E. et al. A human-specific modifier of cortical connectivity and circuit function. Nature599, 640–644 (2021). ArticleADSCASPubMedPubMed CentralGoogle Scholar
- Libé-Philippot, B. et al. Human synaptic neoteny requires species-specific balancing of SRGAP2–SYNGAP1 cross-inhibition. Preprint at bioRxiv 10.1101/2023.03.01.530630 (2023).
- Lucas, B. & Hardin, J. Mind the (sr)GAP—roles of Slit–Robo GAPs in neurons, brains and beyond. J. Cell Sci.130, 3965–3974 (2017). ArticleCASPubMedPubMed CentralGoogle Scholar
- Charrier, C. et al. Inhibition of SRGAP2 function by its human-specific paralogs induces neoteny during spine maturation. Cell149, 923–935 (2012). This study demonstrates that the human-specific geneSRGAP2Cinhibits SRGCAP2A activity, delaying spine maturation. ArticleCASPubMedPubMed CentralGoogle Scholar
- Dennis, M. Y. et al. Evolution of human-specific neural SRGAP2 genes by incomplete segmental duplication. Cell149, 912–922 (2012). ArticleCASPubMedPubMed CentralGoogle Scholar
- Heide, M. et al. Human-specific ARHGAP11B increases size and folding of primate neocortex in the fetal marmoset. Science369, 546–550 (2020). ArticleADSCASPubMedGoogle Scholar
- Florio, M. et al. Human-specific gene ARHGAP11B promotes basal progenitor amplification and neocortex expansion. Science347, 1465–1470 (2015). ArticleADSCASPubMedGoogle Scholar
- Franchini, L. F. & Pollard, K. S. Human evolution: the non-coding revolution. BMC Biol.15, 89 (2017). ArticlePubMedPubMed CentralGoogle Scholar
- Romero, I. G., Ruvinsky, I. & Gilad, Y. Comparative studies of gene expression and the evolution of gene regulation. Nat. Rev. Genet.13, 505–516 (2012). ArticleCASPubMedPubMed CentralGoogle Scholar
- Sholtis, S. J. & Noonan, J. P. Gene regulation and the origins of human biological uniqueness. Trends Genet.26, 110–118 (2010). ArticleCASPubMedGoogle Scholar
- Noonan, J. P. Regulatory DNAs and the evolution of human development. Curr. Opin. Genet. Dev.19, 557–564 (2009). ArticleCASPubMedGoogle Scholar
- Shibata, M. et al. Regulation of prefrontal patterning and connectivity by retinoic acid. Nature598, 483–488 (2021). ArticleADSCASPubMedPubMed CentralGoogle Scholar
- Shibata, M. et al. Hominini-specific regulation of CBLN2 increases prefrontal spinogenesis. Nature598, 489–494 (2021). Refs. 176 and 177 report molecular mechanisms of increased connectivity in the prefrontal cortex in humans. ArticleADSCASPubMedPubMed CentralGoogle Scholar
- Jeong, H. et al. Evolution of DNA methylation in the human brain. Nat. Commun.12, 2021 (2021). ArticleADSCASPubMedPubMed CentralGoogle Scholar
- Kozlenkov, A. et al. Evolution of regulatory signatures in primate cortical neurons at cell-type resolution. Proc. Natl Acad. Sci. USA117, 28422–28432 (2020). ArticleADSCASPubMedPubMed CentralGoogle Scholar
- Xu, C. et al. Human-specific features of spatial gene expression and regulation in eight brain regions. Genome Res.28, 1097–1110 (2018). ArticleCASPubMedPubMed CentralGoogle Scholar
- Babbitt, C. C., Haygood, R., Nielsen, W. J. & Wray, G. A. Gene expression and adaptive noncoding changes during human evolution. BMC Genomics18, 435 (2017). ArticlePubMedPubMed CentralGoogle Scholar
- Vermunt, M. W. et al. Epigenomic annotation of gene regulatory alterations during evolution of the primate brain. Nat. Neurosci.19, 494–503 (2016). ArticleCASPubMedGoogle Scholar
- Reilly, S. K. et al. Evolutionary changes in promoter and enhancer activity during human corticogenesis. Science347, 1155–1159 (2015). ArticleADSCASPubMedPubMed CentralGoogle Scholar
- Shibata, Y. et al. Extensive evolutionary changes in regulatory element activity during human origins are associated with altered gene expression and positive selection. PLoS Genet.8, e1002789 (2012). ArticleCASPubMedPubMed CentralGoogle Scholar
- Zeng, J. et al. Divergent whole-genome methylation maps of human and chimpanzee brains reveal epigenetic basis of human regulatory evolution. Am. J. Hum. Genet.91, 455–465 (2012). ArticleCASPubMedPubMed CentralGoogle Scholar
- Oldham, M. C., Horvath, S. & Geschwind, D. H. Conservation and evolution of gene coexpression networks in human and chimpanzee brains. Proc. Natl Acad. Sci. USA103, 17973–17978 (2006). ArticleADSCASPubMedPubMed CentralGoogle Scholar
- Prescott, S. L. et al. Enhancer divergence and cis-regulatory evolution in the human and chimp neural crest. Cell163, 68–83 (2015). ArticleCASPubMedPubMed CentralGoogle Scholar
- Mangan, R. J. et al. Adaptive sequence divergence forged new neurodevelopmental enhancers in humans. Cell185, 4587–4603.e23 (2022). ArticleCASPubMedPubMed CentralGoogle Scholar
- Hubisz, M. J. & Pollard, K. S. Exploring the genesis and functions of Human Accelerated Regions sheds light on their role in human evolution. Curr. Opin. Genet. Dev.29, 15–21 (2014). ArticleCASPubMedGoogle Scholar
- Pollard, K. S. et al. An RNA gene expressed during cortical development evolved rapidly in humans. Nature443, 167–172 (2006). This study reports on the identification of HARs, the fastest evolving regulatory elements in the genome. ArticleADSCASPubMedGoogle Scholar
- Whalen, S. & Pollard, K. S. Enhancer function and evolutionary roles of human accelerated regions. Annu. Rev. Genet.56, 423–439 (2022). ArticleCASPubMedPubMed CentralGoogle Scholar
- Whalen, S. et al. Machine learning dissection of human accelerated regions in primate neurodevelopment. Neuron111, 857–873.e8 (2023). ArticleCASPubMedPubMed CentralGoogle Scholar
- Pizzollo, J., Zintel, T. M. & Babbitt, C. C. Differentially active and conserved neural enhancers define two forms of adaptive noncoding evolution in humans. Genome Biol. Evol.14, evac108 (2022). ArticlePubMedPubMed CentralGoogle Scholar
- Uebbing, S. et al. Massively parallel discovery of human-specific substitutions that alter enhancer activity. Proc. Natl Acad. Sci. USA118, e2007049118 (2021). ArticleCASPubMedGoogle Scholar
- Girskis, K. M. et al. Rewiring of human neurodevelopmental gene regulatory programs by human accelerated regions. Neuron109, 3239–3251.e7 (2021). ArticleCASPubMedPubMed CentralGoogle Scholar
- Weiss, C. V. et al. The cis-regulatory effects of modern human-specific variants. eLife10, e63713 (2021). ArticleCASPubMedPubMed CentralGoogle Scholar
- Reilly, S. K. & Noonan, J. P. Evolution of gene regulation in humans. Annu. Rev. Genomics Hum. Genet.17, 45–67 (2014). ArticleGoogle Scholar
- Boyd, J. L. et al. Human–chimpanzee differences in a FZD8 enhancer alter cell-cycle dynamics in the developing neocortex. Curr. Biol.25, 772–779 (2015). ArticleCASPubMedPubMed CentralGoogle Scholar
- McLean, C. Y. et al. Human-specific loss of regulatory DNA and the evolution of human-specific traits. Nature471, 216–219 (2011). ArticleADSCASPubMedPubMed CentralGoogle Scholar
- Kostka, D., Hahn, M. W. & Pollard, K. S. Noncoding sequences near duplicated genes evolve rapidly. Genome Biol. Evol.2, 518–533 (2010). ArticlePubMedPubMed CentralGoogle Scholar
- Johansson, P. A. et al. A cis-acting structural variation at the ZNF558 locus controls a gene regulatory network in human brain development. Cell Stem Cell29, 52–69.e8 (2022). ArticleCASPubMedGoogle Scholar
- Keough, K. C. et al. Three-dimensional genome rewiring in loci with human accelerated regions. Science380, eabm1696 (2023). ArticleCASPubMedPubMed CentralGoogle Scholar
- Berto, S. & Nowick, K. Species-specific changes in a primate transcription factor network provide insights into the molecular evolution of the primate prefrontal cortex. Genome Biol. Evol.10, evy149 (2018). ArticleGoogle Scholar
- Florio, M. et al. Evolution and cell-type specificity of human-specific genes preferentially expressed in progenitors of fetal neocortex. eLife7, e32332 (2018). ArticlePubMedPubMed CentralGoogle Scholar
- Aughey, G. N., Forsberg, E., Grimes, K., Zhang, S. & Southall, T. D. NuRD‐independent Mi‐2 activity represses ectopic gene expression during neuronal maturation. EMBO Rep.24, e55362 (2023). ArticleCASPubMedPubMed CentralGoogle Scholar
- Salamon, I. & Rasin, M.-R. Evolution of the neocortex through RNA-binding proteins and post-transcriptional regulation. Front. Neurosci.15, 803107 (2022). ArticlePubMedPubMed CentralGoogle Scholar
- Duffy, E. E. et al. Developmental dynamics of RNA translation in the human brain. Nat. Neurosci.25, 1353–1365 (2022). ArticleCASPubMedPubMed CentralGoogle Scholar
- Li, M. & Larsen, P. A. Primate-specific retrotransposons and the evolution of circadian networks in the human brain. Neurosci. Biobehav. Rev.131, 988–1004 (2021). ArticleCASPubMedGoogle Scholar
- Ibarra, I. L. et al. Comparative chromatin accessibility upon BDNF stimulation delineates neuronal regulatory elements. Mol. Syst. Biol.18, e10473 (2022). ArticleCASPubMedPubMed CentralGoogle Scholar
- Beagan, J. A. et al. Three-dimensional genome restructuring across timescales of activity-induced neuronal gene expression. Nat. Neurosci.23, 707–717 (2020). ArticleCASPubMedPubMed CentralGoogle Scholar
- Tyssowski, K. M. et al. Different neuronal activity patterns induce different gene expression programs. Neuron98, 530–546.e11 (2018). ArticleCASPubMedPubMed CentralGoogle Scholar
- Su, Y. et al. Neuronal activity modifies the chromatin accessibility landscape in the adult brain. Nat. Neurosci.20, 476–483 (2017). ArticleCASPubMedPubMed CentralGoogle Scholar
- Kim, T.-K. et al. Widespread transcription at neuronal activity-regulated enhancers. Nature465, 182–187 (2010). ArticleADSCASPubMedPubMed CentralGoogle Scholar
- Hergenreder, E. et al. Combined small-molecule treatment accelerates maturation of human pluripotent stem cell-derived neurons. Nat. Biotechnol.https://doi.org/10.1038/s41587-023-02031-z (2024).
- Kebschull, J. M. et al. Cerebellar nuclei evolved by repeatedly duplicating a conserved cell-type set. Science370, eabd5059 (2020). ArticleCASPubMedPubMed CentralGoogle Scholar
- Homman‐Ludiye, J. & Bourne, J. A. The medial pulvinar: function, origin and association with neurodevelopmental disorders. J. Anat.235, 507–520 (2019). ArticlePubMedPubMed CentralGoogle Scholar
- Biddy, B. A. et al. Single-cell mapping of lineage and identity in direct reprogramming. Nature564, 219–224 (2018). ArticleADSCASPubMedPubMed CentralGoogle Scholar
- Saunders, A. et al. Ascertaining cells’ synaptic connections and RNA expression simultaneously with barcoded rabies virus libraries. Nat. Commun.13, 6993 (2022). ArticleADSPubMedPubMed CentralGoogle Scholar
- Yuan, L., Chen, X., Zhan, H., Gilbert, H. L. & Zador, A. M. Massive multiplexing of spatially resolved single neuron projections with axonal BARseq. Preprint at Biorxivhttps://doi.org/10.1101/2023.02.18.528865 (2023).
- Benavides-Piccione, R. et al. Differential structure of hippocampal CA1 pyramidal neurons in the human and mouse. Cereb. Cortex30, 730–752 (2020). PubMedGoogle Scholar
- Atamian, A. et al. Human cerebellar organoids with functional Purkinje cells. Cell Stem Cell31, 39–51.e6 (2024). ArticleCASPubMedGoogle Scholar
- Chen, Y. et al. Generation of advanced cerebellar organoids for neurogenesis and neuronal network development. Hum. Mol. Genet.32, 2832–2841 (2023). ArticleCASPubMedGoogle Scholar
- Pellegrini, L. et al. Human CNS barrier-forming organoids with cerebrospinal fluid production. Science369, eaaz5626 (2020). ArticleCASPubMedPubMed CentralGoogle Scholar
- Birey, F. et al. Assembly of functionally integrated human forebrain spheroids. Nature545, 54–59 (2017). ArticleADSCASPubMedPubMed CentralGoogle Scholar
- Rao, A., Barkley, D., França, G. S. & Yanai, I. Exploring tissue architecture using spatial transcriptomics. Nature596, 211–220 (2021). ArticleADSCASPubMedPubMed CentralGoogle Scholar
- Longo, S. K., Guo, M. G., Ji, A. L. & Khavari, P. A. Integrating single-cell and spatial transcriptomics to elucidate intercellular tissue dynamics. Nat. Rev. Genet.22, 627–644 (2021). ArticleCASPubMedPubMed CentralGoogle Scholar
- Buenrostro, J. D. et al. Single-cell chromatin accessibility reveals principles of regulatory variation. Nature523, 486–490 (2015). ArticleADSCASPubMedPubMed CentralGoogle Scholar
- Zhu, K. et al. Multi-omic profiling of the developing human cerebral cortex at the single-cell level. Sci. Adv.9, eadg3754 (2023). ArticleCASPubMedPubMed CentralGoogle Scholar
- Domcke, S. et al. A human cell atlas of fetal chromatin accessibility. Science370, eaba7612 (2020). ArticleCASPubMedPubMed CentralGoogle Scholar
- Pesce, L. et al. 3D molecular phenotyping of cleared human brain tissues with light-sheet fluorescence microscopy. Commun. Biol.5, 447 (2022). ArticleCASPubMedPubMed CentralGoogle Scholar
- Rodriguez-Gatica, J. E. et al. Imaging three-dimensional brain organoid architecture from meso- to nanoscale across development. Development149, dev200439 (2022). ArticleCASPubMedGoogle Scholar
- Michalska, J. M. et al. Uncovering brain tissue architecture across scales with super-resolution light microscopy. Preprint at bioRxivhttps://doi.org/10.1101/2022.08.17.504272 (2022).
- Sarkar, D. et al. Revealing nanostructures in brain tissue via protein decrowding by iterative expansion microscopy. Nat. Biomed. Eng.6, 1057–1073 (2022). ArticleCASPubMedPubMed CentralGoogle Scholar
- Rakotoson, I. et al. Fast 3-D imaging of brain organoids with a new single-objective planar-illumination two-photon microscope. Front. Neuroanat.13, 77 (2019). ArticleCASPubMedPubMed CentralGoogle Scholar
- Krafft, P. R. et al. Etiology of stroke and choice of models. Int. J. Stroke7, 398–406 (2012). ArticlePubMedPubMed CentralGoogle Scholar
- Benavides-Piccione, R., Ballesteros-Yáñez, I., DeFelipe, J. & Yuste, R. Cortical area and species differences in dendritic spine morphology. J. Neurocytol.31, 337–346 (2002). ArticlePubMedGoogle Scholar
- Mikula, S., Trotts, I., Stone, J. M. & Jones, E. G. Internet-enabled high-resolution brain mapping and virtual microscopy. NeuroImage35, 9–15 (2007). ArticlePubMedGoogle Scholar
- Barrett, R. D. H. & Hoekstra, H. E. Molecular spandrels: tests of adaptation at the genetic level. Nat. Rev. Genet.12, 767–780 (2011). ArticleCASPubMedGoogle Scholar
Acknowledgements
The authors thank their colleagues for inspiring scientific discussions. This work was supported by the Netherlands Organisation for Scientific Research (NWO-Rubicon, 019.211EN.032ß) and an European Molecular Biology Organisation non-stipendiary postdoctoral fellowship (EMBO, ALTF 845–2021) to F.W.L.; an Klingenstein-Simons fellowship, a Simons Foundation BTI award, and an NIH BRAIN Initiative grant (UM1MH130981) to F.M.K.; the Gladstone Institutes, Chan Zuckerberg Biohub San Fransisco and the NIMH (U01-MH116438) to K.S.P.; and the Medical Research Council (MC_UP_1201/9) and the European Research Council (ERC-STG, 757710) to M.A.L.